- Academic Editor
The review focuses on the recent knowledge on natural anthraquinones (AQs) of plant origin and their potential for application in an exclusive medicinal curative and palliative method named photodynamic therapy (PDT). Green approach to PDT is associated with photosensitizers (PS) from plants or other natural sources and excitation light in visible spectrum. The investigations of plants are of high research interests due to their unique health supportive properties as herbs and the high percentage availability to obtain compounds with medical value. Up-to-date many naturally occurring compounds with therapeutic properties are known and are still under investigations. Some natural quinones have already been evaluated and clinically approved as anti-tumor agents. Recent scientific interests are beyond their common medical applications but also in directions to their photo-properties as natural PSs. The study presents a systematic searches on the latest knowledge on AQ derivatives that are isolated from the higher plants as photosensitizers for PDT applications. The natural quinones have been recognized with functions of natural dyes since the ancient times. Lately, AQs have been explored due to their biological activity including the photosensitive properties useful for PDT especially towards medical problems with no other alternatives. The existing literature’ overview suggests that natural AQs possess characteristics of valuable PSs for PDT. This method is based on an application of a photoactive compound and light arrangement in oxygen media, such that the harmful general cytotoxicity could be avoided. Moreover, the common anticancer and antimicrobial drug resistance has been evaluated with very low occurrence after PDT. Natural AQs have been focused the scientific efforts to further developments because of the high range of natural sources, desirable biocompatibility, low toxicity, minimal side effects and low accident of drug resistance, together with their good photosensitivity and therapeutic capacity. Among the known AQs, only hypericin has been studied in anticancer clinical PDT. Currently, the natural PSs are under intensive research for the future PDT applications for diseases without alternative effective treatments.
The naturally occurring compounds including anthraquinones (AQs) have been under intensive investigations because of the fast development of drug resistance towards conventional chemo-drugs and routine therapeutics [1]. Moreover, there is a general tendency of back to the nature as a main source of low toxicity substances with comparable biological activity as the synthetic analogues. Worldwide, ~80% plants are used to treat diseases in everyday life [2]. As a result of the recognition of the numerous traditional routines in healthcare systems, these medicinal plants and herbs have increasingly been in free usages. Most of the natural AQ derivatives that have been isolated from plants are introduced to be photosensitive compounds in the ultraviolet (UV)-visible spectrum [3]. Among the natural photosensitizers (PS) only few of them such as chlorophyll, curcumin, pheophorbide A, hypocreline and AQs aloe-emodin, and hypericin have been studied for photodynamic therapy (PDT) method [4]. Nonetheless that most of the natural occurring-PSs have been evaluated with properties apart of being ideal PS, their research and development as photosensitive compounds for PDT are still under research interests because of other advantages of their curative actions [5]. A better solution to the main problem with harsh dark and phototoxicity of synthetic PSs are the photosensitive compounds of natural origin.
Currently, only synthetic PDT drugs are in clinical practice [6]. The properties
of the known AQs such as low water-solubility, insufficient selectivity, long
lasting skin phototoxicity are leading to many complications and undesirable side
effects including genotoxicity and mutagenicity. These drugs are under further
improvement through different structural modifications. It is worth to refer to
the nature for PDT drugs starting with the known as well as the novel natural
photoactive compounds. Among the natural AQs, only hypericin (HYP) found in the
plant species of the genus Hypericum L., especially Hypericum
perforatum L. has already been clinically investigated in cancer PDT [7].
Chemically HYP is a polymeric 1,8- dihydroxyanthraquinone
(4,5,7,4
One of the largest groups of natural quinones that can be found in plants, but also in fungi, insects and lichens are 1,8-dihydroxyanthraquinones [10]. Initially, AQs are discovered as natural colorants with variety of colors that make them usable as dyes and pigments in industrial coloration [11]. Their polycyclic planar, rigid aromatic structure contains 14 carbons in the fused benzene rings which is tricyclic anthracene with two carbonyl groups including two benzene rings. The largest class of quinones are oxidized forms of anthracene derivatives which include 1,4- and 9,10-anthraquinones creating dioxoanthracene with characteristics of natural pigments.
The most studied plants containing AQs are part of mainly three families (81%): Fabaceae, Rubiaceae and Polygonaceae [12]. Additional families that contain AQ-derivatives are Asphodelaceae, Rhamnaceae, Hypericaceae, Scrophulariaceae, Bignoniaceae, Pedaliaceae, Verbenaceae and Valerianaceae. However, in higher amount AQs can be found in Fabaceae (Cassia spp. and Senna spp.), Rubiaceae (Morinda spp. and Rubia spp.), Polygonaceae (Rheum spp. and Rumex spp.), Rhamnaceae (Rhamnus spp. and Berchemia spp.) and Asphodelaceae (Aloe spp. and Asphodeline spp.). Among the most identified AQ-derivatives are aloe emodin, chrysophanol, emodin, physicion, rhein, rubiadin and soranjidiol, and damnacanthal (Supplementary Table 1).
Recent literature reviews suggested that the plants’ occurring AQs possess a broad spectrum of medical actions, including antimicrobial, antiviral, cathartic, anticancer, diuretic, vasorelaxant, phytoestrogen, autoimmune disorders and many other clinical applications [13, 14]. The wavelength of HYP (~590 nm) used for photo-experiments is considered as limitation for PDT due to the lesser light penetration in disordered cells [15]. This determines the development of the synthetic AQs [16]. PDT procedure includes a multi-factorial step that generally lead to production of toxic oxygen species (Fig. 1). The photodynamic compounds aim to produce different reactive oxygen species (ROS) by two mechanisms including oxygen radicals and anions (Type I) and singlet oxygen (Type II). The both are initiated by photon energy and next the electron/ proton or an energy transfer reaction due to formation of excited-state PSs. The produced reactive species further affect the pathologic target cells by several mechanisms [17]. There are limited numbers of studies on natural AQs, which are related to possible mechanisms of PDT action [18, 19, 20]. The PDT response might lead to apoptosis or necrosis, degeneration of the tumor vasculature, stimulation of anti-tumor immune response, and inflammatory reactions in the treated lesion (Fig. 1).
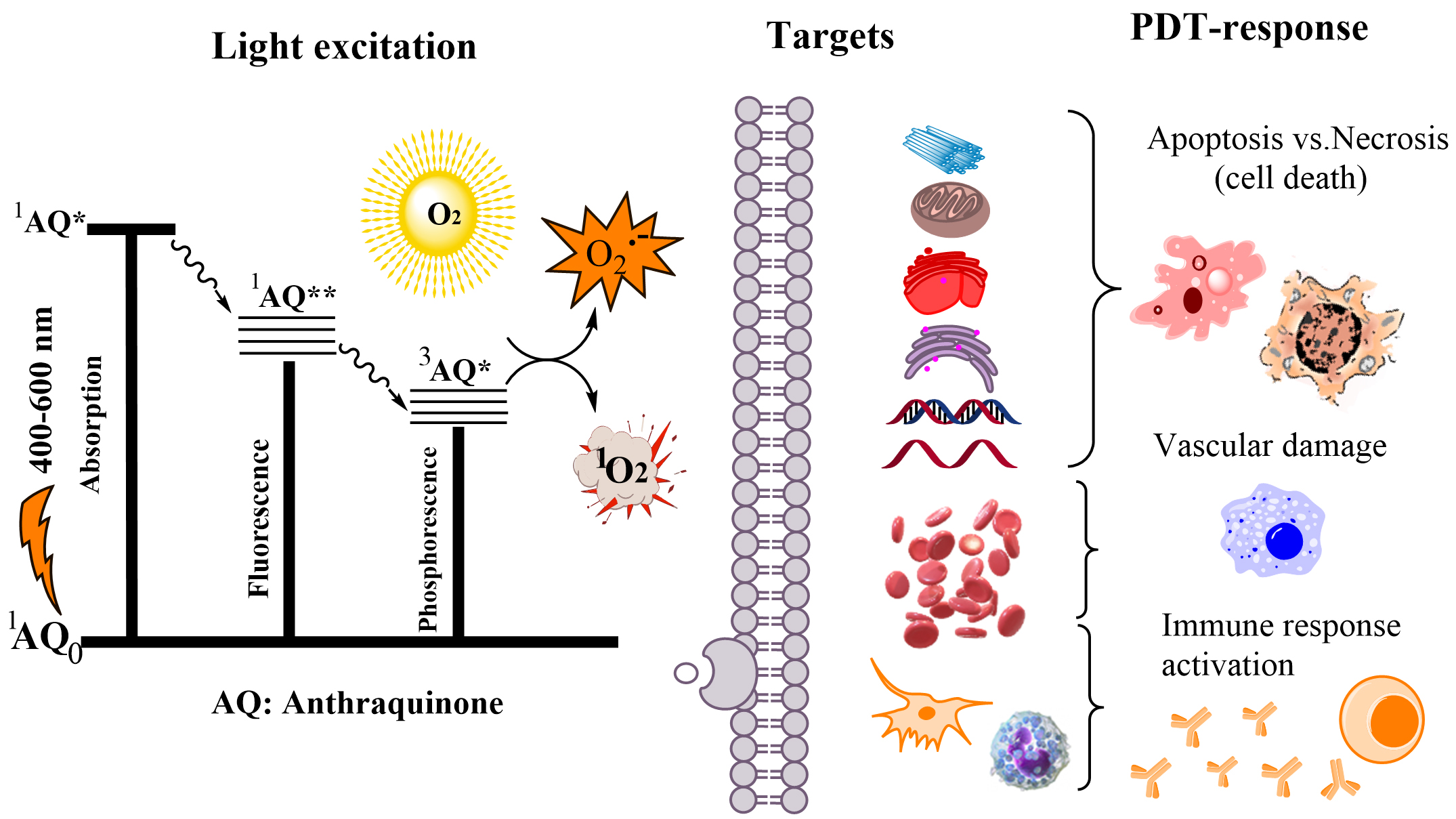
Mechanisms of anthraquinone (AQ) photo-excitation and the possible pathways of the photodynamic therapy (PDT) action and the targets. *: singlet or triplet excited state of AQ; **: lowest energy excited state of AQ.
The plant-occurring AQs are among the natural producers of anthracycline drugs
which are observed as the most effective antineoplastic agents with planar
tetracyclic molecules based on AQ core linked to a sugar moiety and phenolic
groups [21]. The first clinically accepted compounds in cancer chemotherapy were
daunorubicin and doxorubicin (DOX) with DNA binding by intercalation and the
trapping of DNA Topoisomerase II complexes [22]. As a DNA intercalator, DOX forms
hydrogen-bonds with guanine and insert into the DNA strand at sites with adjacent
guanine-cytosine (GC) base pairs. This process tends to cause DNA damage and induce cell death. A
second, Topoisomerase II poisoning by DOX interrupts DNA replication and
transcription, resulting in apoptosis mediated cell death. The oxidation of DOX
consequences in radical formation and then, the superoxide and H
This review summarizes on the recent studies of natural AQs originated from higher plants and the main plants’ families with plant-originated AQs which are already studied as therapeutics. This is an attempt for a critical overview on the AQs as photosensitizers and their phototoxicity and photodynamic action. Additionally, we made a discussion on the chemical structure, and the correlation between the photosensitizing properties and biological PDT functions of the most investigated plants extracts and the isolated AQs. The limited AQ-PDT studies on different pathological targets (tumors, viruses, pathogenic bacteria, fungi and others) are described and discussed in respect to the potential usage of AQs in clinical PDT practice for an alternative curative therapy due to resistance and in emergency cases.
The article is based on the systematic overview on the research papers and reviews published in the last years considering the research field of our interest. The literature sources taken from the PubMed, Scholar Google, Web of Science and Scopus databases are issued during last ten years (till end of 2023) but not limited to this time for some themes. Supplementary Material summarizes in a Table the data about AQs with their structures and the plants of their origin during the first appearance in the literature until the time of manuscript preparation. The searches were made using the keywords “anthraquinones”, “plants and herbs”, “photosensitizers” and “photosensitization”, “photodynamic therapy”, “phototoxicity”. The searches were limited to the most popular AQs isolated from higher plants which are already in usage as medicinal herbs with focus on the photo-properties and PDT studies.
A great number of anthraquinones (AQs) have been isolated from higher plant species and subsequently characterized. Those higher plant speaces are spreading mainly in angiosperm of limited number of families [26]. In plants, fungi, and lichens, there are around 700 representatives species of this group. Compounds with origin from flowering plants which contain AQs as was lately described are approximately 200 structures [27]. Different quantities of AQs have been found in various parts of plant tissues namely flowers, roots, stems, rhizomes, bark, fruits and leaves like rhizomes. In those plants, where the biosynthesis goes by the shikimate-mevalonate pathway, the AQs predominate as aglycones [28, 29]. Much more derivatives of AQs (~700) are described as useful dyes and pigments, making them the most significant group of naturally occurring colorants [30]. Natural AQs are well-studied secondary metabolites, products of biosynthesis that occurs via two major metabolic pathways, namely polyketide pathway (acetate-malonate pathway) and the shikimate pathway. The main difference between the both biosynthetic pathways is that one produces 1,8-dihydroxy anthraquinones (acetate-malonate pathway) and the other (shikimate) pathway never generates substitution at position 8 (Fig. 2). The cathartic activity of some AQs is related to the 1,8-diOH substitution [26, 30]. Anthraquinones from Rubia spp. are produced through the chorismite/o-succinylbenzoic acid or shikimate pathways [31], while the emodin type AQs (among them are the most effective photoactive compounds) are predominantly synthesized through the polyketide pathway [32].
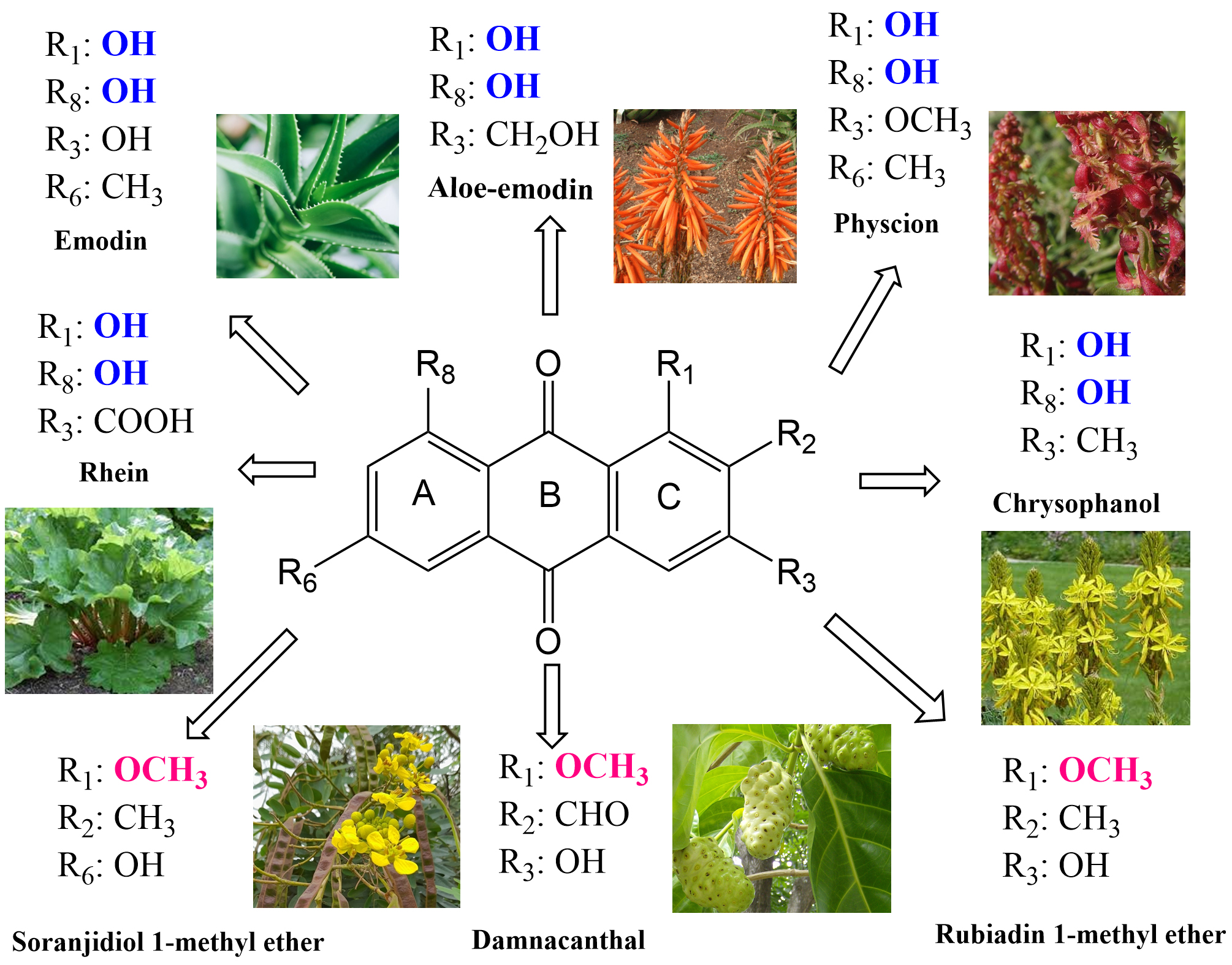
Anthraquinones from higher plants with properties for PDT. Their common substitution groups are in color and close to the plants.
The plants of the genus Cassia L. are an important source of naturally occurring AQs mainly used as bioactive compounds with antifungal, antibacterial, antiviral, insecticidal and other biological activity [33, 34]. A large number of AQ structures were isolated from various parts of the Cassia spp. [35]. The numerous derivatives of AQs are characterized in these plants which are widely used in traditional folk medicines as therapeutics in antimalarial, ulcer healing, anti-diabetic, hepatoprotective, nephroprotective, antitumor and also in treatment of skin infection from the population over the tropical and subtropical regions [36, 37, 38]. Firstly, the plant extracts have been progressively used not only for cosmetics, foods and dyes but also for treatment of different medical problems and acute diseases due to their wide therapeutic and pharmacological properties [39, 40]. Many Cassia spp. exist worldwide that are mostly used in herbal medicine [41]. These particular family of plants is known for their laxative actions. Cassia fistula L. has the capacity of curative important and/or nutritionally valuable source. Leafs of Cassia fistula mainly contain AQ-derivatives together with other group of compounds (quinones, oxalic acids and tannins). The phytochemical investigation reveals that this specie contains a mixture of chrysophanol, physcion, rhein and other non-AQ compounds [42]. The reduced toxicity and minimal side effects of natural AQs observed in Cassia plants feature their potential as therapeutics. A recent report of Oliveira et al. [43] showed the first-time successful application Senna macranthera I&B., Senna splendida I&B. and Senna alata L. leaves and branches extracts as photoactive substances to treat pathogenic bacteria of Cutibacterium acnes, Candida albicans, Staphylococcus aureus and Streptococcus mutans. The study showed seven AQs and one naphthopyrone as potential photoactive substances in the extracts of liquid chromatography –diode-array detection-mass spectrometry (LC-DAD-MS) analysis [43]. The data about the microbial resistance and the treatment of viral infections including COVID-19 are in an early stage of the studies [44]. The investigation of the crude ethanolic plant extracts of Albizia procera (Roxb.) Benth. (Fabaceae) and Lumnitzera racemosa Willd. (Combretaceae) showed that they are non-toxic on cancer but also on non-cancer cells [45]. However, the irradiation of the same extracts demonstrated photocytotoxic effect against breast cancer cells (MCF-7).
Rubiaceae family contains majorly terrestrial plants including more than 13,000 species which are distributed in at least 650 genera. This family belongs to the Gentianales, which worldwide spreading makes it the fourth largest angiosperms. Rubiaceae contains a large amount of AQ derivatives, particularly in the roots [46]. The various parts of the species Morinda elliptica L. followed by Heterophylleae pustulata Hook. f. and Morinda royoc L. are also rich source of AQs. These very important natural products have been evaluated with various bioactivities, including antibacterial, antiviral and antitumor, and antiparasitic which make them of high interests for the research studies [47]. At the present, the increasing incidence of terminal infections caused by antibiotic-resistant bacteria tends the discovery of new drugs originated from natural substances with wide-spectrum of antimicrobial action [48].
Many Chinese herbs namely Rheum palmatum L., Fallopia
multiflora Harald. and Reynoutria japonica Houtt. are source of AQs
including aloe emodin, emodin and other biologically-active ingredients [49].
This study concluded that the development of traditional Chinese medicine based
on AQs is prospective in combating the drug resistant pathogenic bacteria. The
antibacterial effects of emodin, rhein and aloe emodin are more potent than those
of emodin methyl ether and chrysophanol [50]. Structurally, these AQs have the
same parent nucleus but they differ from their substituents on positions 3 and 6
(Fig. 2). The polar substituents –COOH and –CH
Rubiaceae family of flowering plants have been reported to have a phototoxic effect under the sun-light exposure, being part of animal feedstuffs. H. pustulata (Rubiaceae) grows in the Andean mountain range of the northwest of Argentina, and the plants were evaluated with strong undesirable phototoxicity [51, 52]. The phototoxicity of rubiadin and soranjidiol which were mostly found in the aerial parts of H. pustulata was observed on the animals after consuming the plant and staying on sunlight [51]. At skin level the observed effects were inflammation, erythema, itching and petechiae but also some changes of the behavior such as nervousness, aggressiveness and photophobia. The isolated AQs rubiadin 1-methyl ether, damnacanthal, soranjidiol, pustuline, damnacanthol, and heterophylline were evaluated with photosensitizing properties due to generation of superoxide anion under illumination [52]. The control group of animals kept in dark place showed neither anomalous behavior nor skin phototoxicity as well as the control group of animals exposed to light. The metabolic species were evaluated with generation of radical/ions typical for photosensitization reactions. In view of the toxicity the latest known benefit is the low systemic toxicity of AQs, which is just the opposite observation on the previous finding (excluding danthron) [51, 52]. There are negligible side effects due to everyday light exposure as might be not observed for synthetic drugs.
Chemical investigation of the plant leaves and stems of H. pustulata revealed the numerous AQs (9,10- anthraquinone derivatives),
soranjidiol, soranjidiol 1-methyl ether, rubiadin, rubiadin 1-methyl ether,
damnacanthal, damnacanthol, heterophylline, pustuline, 2-hydroxy-3-methyl
anthraquinone and (S)-5,5
The plant H. pustulata was evaluated with photoactive AQs
rubiadin and soranjidiol that have different biological activity. In case of
monoculture of colon cancer cells, two AQs such as soranjidiol and rubiadin
showed no significant difference in the photosensitizing ability as they did in
spheroid cultures [57]. It was determined that a concentration of 10 µg/mL
for rubiadin and soranjidiol retains the cellular viability up to 80% in
mammalian eukaryotic Vero cells [58]. The study described that cells cultures did
not deliver soranjidiol and rubiadin into the cellular nucleus, which is
presenting a minimal mutagenesis risk. More so after irradiation the generation
of ROS was considerably and consistently higher for the treated cells and this is
one of the desirable behaviors for PSs. As well, both AQs exist as regioisomers
formed during the biosynthetic shikimic acid pathway (acid
o-succinilbenzoico; mevalonate) [59]. Photosensitizing rubiadin,
soranjidiol, damnacanthal and 5,5
As is known the photocytotoxicity depends on the proper interactions between PS,
light and oxygen, but the first object is the chemical structure of a PS, which
further determines the light penetration depth and in the end the oxygen
availability [62]. The oxygen atmosphere is critical for the sufficient
production of ROS and other species which are needed to induce cells
inactivation. The mechanism of ROS generation is typical for the photosensitive
natural AQs [63]. Another study shows for the first time the reduction of
biofilms of Candida tropicalis with two AQs [64]. More effective
inactivation was observed with rubiadin (AQ1) than with rubiadin 1-methyl (AQ2).
The mechanism of action after light application was examined as mediated by an
oxidative and nitrative stress with formation of endogenous ROS mainly
O
Polygonaceae plants have a worldwide distribution, most of which are in the temperate region of the Northern Hemisphere. There are 235 Polygonaceae species and 37 varieties in China. Plants of the Polygonaceae family have been shown with a high frequency of AQ structured compounds. The Polygonaceae are commonly known as the knotwood or smartweed family, which consist of flowering plants and comprise about 1200 species in 48 genera [66]. Among which the largest ones are Eriogonum Michx. (2410 spp.), Rumex L. (200 spp.), Cocoloba P. Browne (120 spp.) and Persicaria Mill. (100 spp.) and among others. This family is wildly distributed in the North Temperature Zone although can be found worldwide [67]. The best known compounds emodin, rhein, physcion, chrysophanol, catenarin, and pupurin have been studied to have a wide range of versatile bioactivities, including bactericidal, fungicidal, antioxidant, anti-inflammatory, anticancer, and many others attributed to substances in this family [68, 69].
The well known compounds such as emodin, chrysophanol, and aloe emodin have been isolated from different Polygonum spp., including Polygonum bistorta L. [70]. A potential antiviral activity of emodin isolated from Rheum palmatum L., Rheum tanguticum Balf., Rheum officinale Baill., Polygonum cuspidatum Siebold & Zucc. and Polygonum multiflorum Thunb. was reported in a recent review [13]. A traditional Chinese medicine plant P. cuspidatum has roots rich in three AQs namely emodin (50 µg/mg), physcion (10 µg/mg) and rhein (5.6 µg/mg) [71]. The results included a visible light-dependent decrease of HSV-2 plaque using the extract for inactivation of Herpes virus. The photosensitizing effect of extract was due to emodin as the main active compound with a high production of ROS while no reduction effect was observed for the control samples without light irradiation.
Many different genera of Polygonaceae family including Rheum spp., Polygonum spp. and Rumex spp. have been documented to contain AQ-derivatives with phototoxicity action [72]. Importantly, the antiviral activity was demonstrated to be a combination of effects of different classes of compounds with or without photoactivity. So that they can have a potential in the development of new photo-active drugs [73]. The studied AQs from the plants of the above families have shown their effects against viruses that infect the respiratory tract, including human respiratory syncytial virus and influenza virus which infect the respiratory tract like coronavirus. AQs were evaluated with high capacity to inhibit coronavirus in vitro and in silico which could serve as a base for development of effective antiviral drugs towards coronaviruses [74].
The photodynamic activity studied for aloe emodin (AE) isolated from
Rheum palmatum L. and Aloe barbadensis Miller against
pathogenic bacteria and fungi showed a high efficiency of this compound [75, 76, 77].
This study suggested that the limitation of AE absorption spectrum leads to PDT
effectiveness only for treatment of superficial diseases (skin cancers,
infections in oral cavity, eye conditions and among others). The singlet oxygen
quantum yield (
Asphodelaceae is a family of monocotyledonous flowering plants occurring in
Africa and spread from the Mediterranean to central Asia. The genus
Asphodeline Rchb., containing 14 species, common in Mediterranean region
from this family and subfamily Asphodeloideae. Three of them specifically,
A. lutea (L.) Reichenb., A. liburnica (Scop.) Rchb,
and A. taurica (Pall. ex M.Bieb.) Endl. are growing in Europe
Balkan region. The main gene Asphodeline includes 20 taxa of which 12
are endemic in Anadolu region [83]. The Asphodeline spp. are
traditionally used as medicinal plants in Turkish folk medicine and for human
diet. These are A. damascena subspp. damascena and A.
tenuior subspp. tenuiflora with applications for healing of scratches
and verrucae in traditional medicine [83]. The studies showed that the roots from
Asphodeline spp. are rich source of both monomeric chrysophanol and
dimeric asphodeline with probable photosensitizing effect [84]. In addition, many
other AQs such as aloe-emodin, isochrysophanol, physcion, rhein,
1,5,8-trihydroxy-3-methylanthraquinone, 2-acetyl-1-hydroxy-8-methoxy-3
methylanthraquinone, asphodeline and 1,1
The most studied valuable genera belonging to Asphodeloideae subfamily is
Aloe vera L. and the roots of Aloe spp. are containing
1-methyl-8-hydroxyanthraquinones [88]. The extracts were shown to be protective
against photodamages after removing the fraction with AQ [89]. The major
bioactive compound in the leaves and roots of Aloe vera L. is AE which
has been studied with a broad spectrum of anti-cancer activity [90]. The
photochemical activity of AE was evaluated with phototoxicity against the K3 cell
line with an increased effect (77%) due to irradiation with blue light (405 nm,
12.8 J/cm
Liu et al. [94] reported inhibition of human oral squamous cell
carcinoma in vitro and in vivo, after application of AE-PDT.
The authors concluded that AE-PDT can enhance the killing effect of KB cells
(human epithelial carcinoma cells), inducing the cell apoptosis and finally
leading to cell death in vivo and in vitro, hence it was
evaluated as an effective therapy to cure oral cancers. It demonstrates that cell
apoptosis rate in PDT is apparently higher than other single therapeutic
approaches. PDT is much more effective in tumor growth inhibition and the
retention of AE in tumors after treatment may promote KB cell apoptosis. This
study concluded that AE mediated PDT is an innovative approach for oral cancer
treatment with the supremacies of effectivity, minimal invasion, tissue integrity
retention and none observed side effects on main organs. The light exposure in
presence of oxygen was shown to endorse photochemical reaction including the
generation of singlet oxygen but also in high extend many other ROS which can be
in advance for treatment of hypoxic pathological conditions such as malignant
tumours and resistant air-free bacterial species [92, 93, 94]. The mechanism of cell
death is associated with inducement of cell cycle arrest with an increment of
apoptosis by upregulation of Caspase-3 and Bax proapoptotic proteins [95].
Another study on the therapeutic effect of aloe-emodin in nano-liposome (AE-L)
mediated PDT showed a significant killing effect on Tca-8113 cells of human
tongue squamous cell carcinoma [96]. The results indicated that for
concentrations less than 7.5 µg/mL there was no significant difference
between PDT group and control group (p
The genus Rhamnus L. belongs to the Rhamnaceae family contains
approximately 137 species, traditionally used in folk medicine of East Asia,
North and South America, and subtropical regions of Africa. The selected species
Rhamnus purshiana DC., Rhamnus frangula L., Rhamnus
catartica L., Ventilago maderaspatana Gaertn. have usually been used in
treatment of cancer, wound, jaundice, hepatitis, gonorrhea, constipation,
hypertension, malaria, stomach ache, snake bite and diarrhea [98]. Rhamnaceae
family commonly known as a Buckthorn Family consists of ~50
genera and ~900 flowering species, mostly trees, shrubs, and some
vines, and one herb. The family plants are distributed worldwide, especially in
the subtropical and tropical origins [98]. The pharmacological analysis showed
that the extracts and isolated several AQs found in the Rhamnus spp.
revealed different biological activity as potential photosensitizers. The
derivative prinoidin isolated from the fruits of R. nepalensis Laws.
(Rhamnaceae) have four times more potent cytotoxic effect against human
epidermoid carcinoma with IC
The natural photosensitive compounds such as chlorophylls and chlorophyll metabolites, curcumin, thiophenes, alkaloids, as well as anthraquinones have been featured of importance for investigations as photosensitizers for PDT applications (Fig. 3). Recent work about the phototoxic terrestrial plants including the plants containing AQs, reviews the phototoxic and photosensitizing metabolites of 250 vascular plants that have been documented as phototoxic or with photosensitive properties [100]. This review presents an in-depth investigation with the list of phytochemicals synthesized by terrestrial plants with the photosensitive compounds, and the basic molecules for the further development of new PDT-PSs. The compounds originated from different plant species are under intensive research due to their promising photocytotoxicity in the treatment of cancer and microbial infections [101]. This indicates the future for natural compounds among the class of AQs as anticancer and antiinfection therapeutics (Fig. 3). Moreover, PDT is recognized as a method with a high impact on the resistance inhibition.
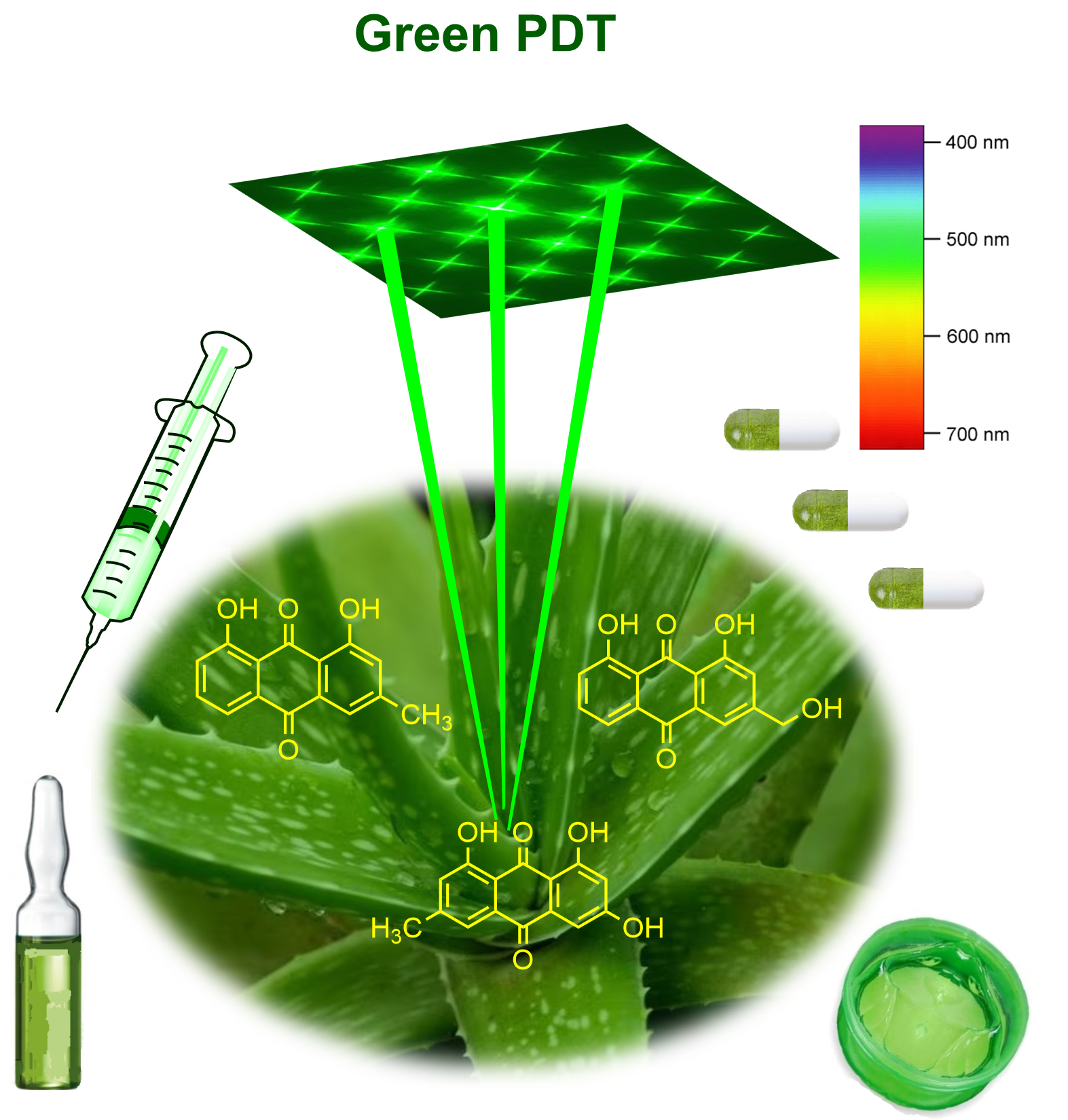
Green PDT approach due to the spectra and plants’ origin of the photosensitive anthraquinone compounds.
Presently, the application of quinones as PDT agents is limited because of their
intensive absorption in the whole UV spectrum, the lack of selectivity, fast
clearance and strong hydrophobicity. However, there are few derivatives that can
be used as potential PDT agents after properly performed structural modification,
so that the absorption relocates to the longer wavelength (
Currently, several chemotherapeutic anticancer agents containing AQ-nucleus are the Food and Drug Administration (FDA) approved drugs, namely daunorubicin, idarubicin, doxorubicin (DOX), epirubicin, valrubicin, pixantrone and mitoxantrone all for clinical use in cancer therapy [106]. The appearance of drug resistance and some possible side effects as for example, the heart muscle injure after DOX treatment, lower the use of doxorubicin-based cancer treatments. Mechanisms of antitumor action of these anthracycline drugs are associated with hyperproduction of ROS and inhibition of glycolysis [107]. However, there is an emerging limitation related to the poor efficacy of chemotherapeutic agents, due to the resistance of tumor cells to these drugs, as well as due to toxicity-related side effects [108]. The score of new drug discovery is known as very low with the estimation that only ten of ten thousand novel tested compounds have been passed the regulations to enter clinical stage, and then only one may be approved through regulatory valuation and licensed for the medical application. The biologically active substances require a long term and complicated procedure including many stages of research, pre- and clinical trials on humans to develop medicine for the pharmacy shelf [109].
Gollnick et al. [110] (1992) showed in an initial study on AQs that the compounds bearing hydroxyl groups in their structure which are distributed on the benzene rings of both sides may have promising potential for photodynamic action. The further study on the photosensitizing properties of some natural AQs isolated from leaves and stems of Heterophyllaea pustulata Hook. f. (Rubiaceae) showed the both mechanisms of photosensitization for the studied rubiadin, soranjidiol, soranjidiol-1-methyl ether, damnacanthal and damnacanthol [111]. The photosensitized generation of superoxide anion radical (Type I mechanism) was presented only for rubiadin-1-methyl ether as was determined in leukocyte suspensions. The singlet oxygen generation (Type II mechanism) was studied in solution for damnacanthal (0.70) followed by soranjidiol (0.47) which were evaluated with relatively very high yields.
Although there are drugs with AQ structure that have already been approved for clinical practice, a large number of AQ analogues have been synthesized and the synthesis continued [112]. The structure of AQ is suitable for metal/non-metal coordination, but there is a limited number of studies on synthetic AQs prepared as a complexe [113]. The coordination of metals was studied with the synthetic ligands through the carbonyl group at AQ-ring and nitrogen atom of amide group. The antibacterial inactivation of both Gram-positive and Gram-negative pathogenic bacterial species showed the high activity for complexes types [Cd(L1)2Cl2] and [Cd(L2)2Cl2]. The clinical AQs have been found to have positive cardiotoxicity, but after the formation of complexes with metals such as Cu, Co, Fe and Mn was shown to limit the ROS production and reduce their toxic and side effects [114]. This can be a starting point for design of novel AQ complexes with low side effect and the superior mechanism Type II photosensitization for PDT.
A very recent study summarized the phototoxic effect of AQs and the plants of their origin and the compounds that are with promising efficiency on different experimental cancer cells [115]. The research and review papers present the AQs such as emodin, aloe-emodin, parietin, rubiadin, hypericin, and soranjidiol as photosensitive compounds for cancer PDT [116, 117].
Generally, depending on drug and light administration conditions, the PDT effect induces the cell death through several mechanims, which can be the initiation of apoptosis, autophagy-associated cell death or necrosis [118, 119]. Another possible mechanism could be an immunogenic cell death (ICD) [120]. A recent study on aloe emodin (AE) from Rheum palmatum’s rhizome reported a mechanism of the PDT of apoptosis in target cells [121]. The mechanisms of cell-death have been mostly investigated for the natural hypericin [122]. Hypericin shows absorption with maximum close to therapeutic region (630 nm–850 nm), which by the irradiation leads to generation of ROS as well as singlet oxygen. In cells, HYP primarily accumulates in the hydrophobic core of the membrane by forming non-covalent persistent interactions and the lipid double bonds, plus in the endoplasmic reticulum (ER), mitochondria, lysosomes and Golgi apparatus [123]. Recently, Kessel reported the capacity of HYP to target the ER for photodamage, which provokes a mainly unexplored manner of photokilling that implicates extensive cytoplasmic vacuole formation but does not represent autophagy [124]. This phenomenon called ‘paraptosis’ seems to be a response to the appearance of misfolded ER proteins as important to eradicate the malignant cell population with a reduced apoptotic pathway [125, 126].
A review paper about “anthraquinones as a promising scaffold for the discovery
and development of therapeutic agents in cancer therapy” concluded that
in silico approaches (molecular modeling and docking studies) have been
recognized as an extensively useful support for progress of novel anticancer
compounds based on AQ-moieties [127, 128]. The knowledge on the binding
interactions of AQs with amino acids located in active site pocket of targeting
enzymes such as CK2
The conformational analysis with three-dimensional quantitative structure-activity relationships (3D-QSAR) of compounds and thus the design of new
synthetic photosensitizers could provide a methodological reference for the
design and synthesis studies of novel photosensitizers [130, 131]. The AQ moiety
provides a potential substrate for DNA insertion and bio-reduction activation,
and the alkylation group confers cytotoxic activity [130]. DNA intercalators that
typically contain coplanar aromatic backbones such as doxorubicin, daunorubicin,
and mitoxantrone (MTZ) can be reversibly inserted into base pairs of DNA. The
scientists reported that AQ moieties may serve as a base for preparation of
synthetic hydroxyanthraquinone derivatives as the recently reported with
N-mustards, thiophene and benzene ring as substituents. The molecular
docking studies of compounds demonstrated that the new compound (A1) could
interact with the catalytic active site in topoisomerase II. The recent paper
studied five pH-responsive anthraquinone photosensitizers (A1 to A5) synthesized
through addition, elimination, and substitution mechanisms using chrysophanol as
the raw material [131]. The compounds were shown to have good PDT antibacterial
activity and selectivity in weak acid environments. The cytotoxicity (3-[4,5-dimethylthiazol-2-yl]-2,5 diphenyl tetrazolium bromide, MTT test)
on the cancer cell line HeLa demonstrated the photo- and dark toxicity effects of
compounds (for A3 at 0.625 µM) similar to the reference photosensitizer
Chlorin e6 (Ce6). Nevertheless these findings, it is still very questionable to
find a very powerful PS among the plants-originated AQ-compounds because of the
spectral properties of short-wavelength absorption (
Green approach to PDT includes the PS isolated from plants or other natural source and the specific visible light spectra (400–600 nm), and sufficient oxygen surrounding [132]. Presently, there are no clinically approved plants isolated photosensitizers (PSs) for application as drugs in medical PDT. It is only hypericin which pass throughout many years of research and development and pre-clinical studies so that it has been featured with good perspectives for clinical applications [133]. The scientific society expect that it is the near future when the natural PSs and their synthetic analogues to be invented in cancer PDT. The natural AQs can serve as a core molecule for design of novel more powerful anticancer agents with photosensitive properties that are tailored for PDT.
Practically the natural compounds exhibited the poor solubility, high body clearance rate and restricted tumor targeting capability together the expected lower efficiency as PDT drugs. In order to solve these problems, natural PSs and their derivatives might be involved in molecular assembly technique to enhance uptake, selectivity and PDT efficacy in addition to minimal toxicity in the presence of visible light as the main advantage of these PSs. Also, since natural compounds are usually universal, and some of them are already in usage as herbs and therapeutic drugs, they may be more readily-accessible and easy for therapeutic invention.
In future, researchers may discover these photoactive plants in nature and compare the effects with clinical drugs. Furthermore, owning on the well-accepted structures of PSs for PDT, the natural AQs’ might serve as a template for development of variety of novel target-specific PSs containing definite functional groups as substituents for the target specific PDT. The novel compounds may have the tunability of the photochemical and the photobiological properties, high biocompatibility, and selective cellular uptake in the tumors or other target pathologic tissue and cells, and not the last the better solubility in natural fluids, and absorbance in the optical PDT window (630–850 nm). In addition, the far-red fluorescence emission will be advantageous for the non-invasive optical diagnosis.
As researchers, we must consider the enquiry whether or not is realistic the anthraquinone—associated PDT to get permission for clinical practice? The crucial aspect about plants’ PS is that they have not characteristics of typical photosensitizers due to the natural exposure to sunlight which could be harmful and destructive during the plants growing. However, there are good opportunities for natural anthraquinone hypericin, as it is already on clinical level in cancer PDT. Since the progress in PDT involves the applications of the method to the actual diseases due to resistance to present drugs and for the other diseases without alternative treatments or with insufficient treatment consequence. The decisive stage for further development of natural plant-occurring AQ for PDT applications is to find the unique plant or plants that are having high quantity appropriate compounds and substances with valuable photosensitizing properties. This fact will allow to develop a procedure to obtain the photoactive compound(s) in a large scale. Moreover, the plant cultures hold great potential for the manufacture of phytochemicals to obtain natural compounds on a large scale, in an economically viable and eco-friendly manner.
AQ, anthraquinones; AE, aloe emodin; HYP, hypericin; ROS, reactive-oxygen species; LED, light-emitting diode; PS, photosensitizers; PDT, photodynamic therapy.
VM designed, made the searches, wrote original draft, made the corrections, supervised, and answered the reviewers. DB made the searches, wrote Supplementary, prepared original Figures and made critical corrections. IL made searches, wrote paragraphs, and reviewed. TG made searches, wrote draft, and made the critical corrections. All authors read and approved the final manuscript. All authors have participated sufficiently in the work and agreed to be accountable for all aspects of the work.
Not applicable.
Thanks to Bulgarian Academy of Sciences.
This research received no external funding.
Given her role as Guest Editor, Vanya Mantareva had no involvement in the peer-review of this article and has no access to information regarding its peer review. Full responsibility for the editorial process for this article was delegated to Jen-Tsung Chen. The authors declare no conflict of interest.
Publisher’s Note: IMR Press stays neutral with regard to jurisdictional claims in published maps and institutional affiliations.